The next generation of femtosecond workhorses
The next generation of femtosecond workhorses
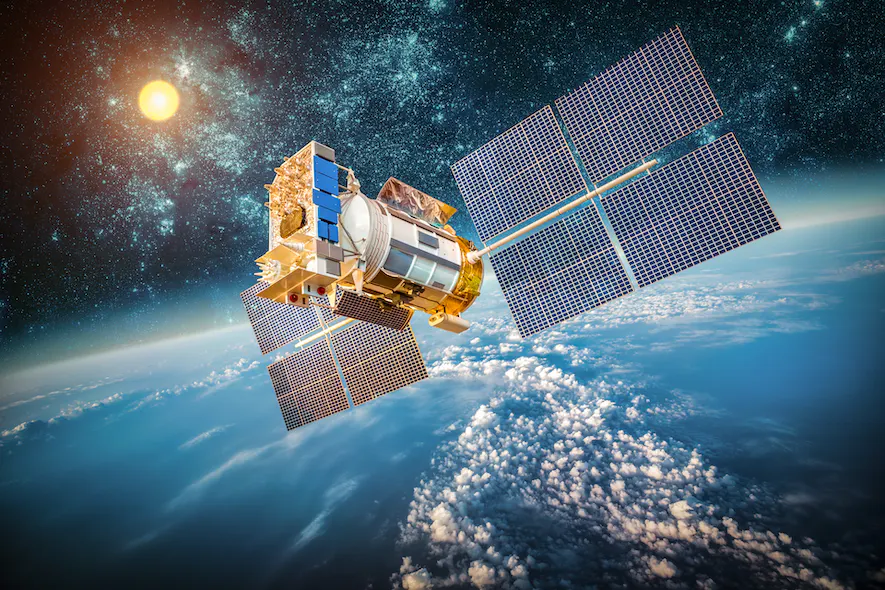
FIGURE 1. In the near future, tiny, rugged femtosecond lasers could serve as a time standard or source for a radio-frequency (RF) signal on satellites and airplanes.
Femtosecond lasers have enabled groundbreaking research since the 1980s. But their development is progressing and more and more applications become possible. The actual strength of newer ultrafast lasers is the extreme stability of their laser pulses. The application-relevant timing jitter for these lasers is much smaller than 1 fs, which is critical for the very challenging application of distributing timing information, such as gigahertz scopes, communication lines, and precision radar.
The development of femtosecond lasers took several decades, and for much of that time they have been the workhorse of scientific research. Those systems were most often based on titanium sapphire (Ti:sapphire) crystals. They were rather sensitive devices, but have also enabled groundbreaking research since the 1980s, earning Nobel Prizes in 1999 and 2018.
Within the last 20 years, we saw the advent of industry-grade systems with turnkey operation. Many of these rely on fiber technology—which can provide stable operation for long periods of time, but still has limitations. More recently, monolithic oscillators were introduced by Menhir Photonics, a Swiss company that is a spinoff from ETH Zurich. The company’s monolithic oscillators offer unprecedented stability and performance and currently come in a shoebox-sized case, but have the potential to reach matchbox size or smaller. And these lasers offer another novel quality: They can be delivered via a regular mail service and run turnkey afterwards.
‘A solution seeking a problem?’
While this quote from Ted Maiman might have been right for the first generation of lasers, it certainly does not hold for the most recent one. The actual strength of newer ultrafast lasers is the extreme stability of their laser pulses. The application-relevant timing jitter for these lasers is much smaller than 1 fs, which is critical for the very challenging application of distributing timing information. For example, these lasers are used at several large telescope sites where signals must be coupled from different telescopes with precise timing into a single image. The same holds for radar applications: Using a precise timing signal distributed via a conventional commercial optical fiber, these systems can couple signals from different sources together, allowing them to either look further or with more detail.
Particle accelerators use these precise timing signals to trigger their systems at the exact right moment. Just imagine particles circling in an accelerator tube at almost the speed of light (300,000 km/s)—in the span of a femtosecond, they will move 0.3 µm. Low-jitter laser signals can be distributed via fibers to any accelerator stage and to any measurement device, giving the right signal at the right moment.
So far, so good, but these are all niche markets. What about larger ambitions?
Analog-to-digital conversion
Throughout the last 60 years, the laser has seen several technological pushes. The biggest came from telecommunication. Here, the introduction of laser diodes, low-loss optical fibers, and, later, erbium-doped fiber amplifiers has led to two effects. The first was a revolution in society through the advent of the internet. The second was a push in electronic and photonic technologies beyond the imaginable. The demand of this huge market accelerated the development of lasers and optical components. Giga- and terabit transmission lines are a major result. Kilowatt fiber lasers, which have taken the lead in materials processing, are actually a nice byproduct of this trend.
Telecom is a big market, and demand for ever more bandwidth is acute. In response, transmission rates have grown beyond gigahertz rates—which is a problem for testing technology, as it is based on electronics. And electronic testing becomes expensive or simply impossible if timing goes deep into the picosecond range. A 1 GHz oscillation has 1000 ps to complete a cycle. To measure this cycle with a resolution of 100 points per cycle requires a resolution of 10 ps. Measuring this signal means turning a detector on at a precise moment and turning it off after a defined time—within a 10 ps window. As such, both detector operations should happen with a precision much better than 10 ps.
Such a precision is achievable with a properly timed laser signal (see Fig. 2). A high-frequency signal that needs to be digitized is used to drive an electro-optical modulator (EOM). The EOM attenuates a laser signal with a high repetition rate, which turns the continuous analog signal into a set of well-separated peaks. These peaks are converted into digital signals by a fast electronic analog-to-digital converter (ADC). Because the ADC is triggered by the original laser signal, it is inherently perfectly timed with regard to the signal that needs to be converted. The laser signal typically has a jitter of less than 1 fs, which is much smaller than the distance between two peaks (two measurement points).
The duration of each peak is defined by the duration of the laser pulse (which is well below 1 ps). This is much smaller than the length of time between two pulses. Therefore, the measuring time of the ADC is less important as long as it does not cut away parts of the pulse. In such a way, the laser improves the signal quality because the aperture jitter no longer depends on electronic properties, but on the length of the laser pulse. The amplitude stability of a MENHIR-1550 laser is better than 0.01% over 109 subsequent pulses, which improves the signal quality as well.
For high sampling rates, time-division multiplexing can be applied via a single 1 GHz laser clocking multiple ADCs, with all ADCs receiving time-shifted trigger signals. Using 100 ADCs, one can get the equivalent of a 100 GHz sampling rate, allowing the measurement of signals up to 50 GHz. While this is the simple explanation for a simple photonic ADC, there are many techniques for more complex photonic ADCs. These will support the next generation of telecom platforms targeting 800 Gbit/s, or even 1.2 Tbit/s.
This is an attractive approach for future sampling scopes used for testing in telecom and datacenter networks. Since this technology is space-tested, it may also be an attractive solution for future satellite communications. Photonic ADCs could significantly reduce the difficulties of signal processing in space.
Microwave signal generation
Measuring precisely is one thing, but these laser systems can generate radio-frequency (RF) signals with low noise and low jitter as well. RF sources in the range of megahertz to gigahertz frequencies have widespread uses. There is radar, of course, but also GPS and many wireless communication methods such as 4G and 5G.
These applications receive their clock signals from electronic oscillators, mainly relying on quartz crystals. Mode-locked lasers producing 10–100 fs optical pulses can serve as sampling clocks that can be 1000–10,000X lower in jitter compared to their microwave-driven counterparts that have a typical period of 100 ps and otherwise similar parameters.1 There are tricks such as cryogenic cooling to make the quartz clock more precise, but they come at the price of cost and size.
Behind all of that is a nice piece of laser physics. The ultrashort-pulsed laser is mode-locked, which means all modes are locked in a way that the sum of all the modes in the laser resonator produces that short pulse. The modes are standing waves; the one with the smallest frequency or largest wavelength is exactly as long as the resonator. All other modes are integer multiples of this basic mode frequency. If we look at this in the frequency space, these modes are equally spaced peaks with a distance of that basic mode frequency—which, quite nicely, equals the repetition rate of the laser resonator. And, because of this efficient process of mode-locking, all these modes are coherent, so they have a fixed phase relation. If we look at this in the spectral or frequency space, then we see what has become well known as a frequency comb.
While the distance between the lines is defined by the laser cavity length, the actual spectral position of such a frequency comb is mainly defined by the laser gain medium. So, there will be a center frequency (or center wavelength) around which the modes will be strongest.
If the different modes are brought to interference on a photodiode, they form a pattern in the frequency domain with all the multiples (or harmonics) of the fundamental frequency. We get a signal that includes all multiples of the repetition rate, with each RF tone being phase coherent to each other. With electronic filtering, a desired multiple of the basic frequency can be derived. While the laser source could generate frequencies up 650 GHz or more, the bandwidth of the detector acts as a filter with a high-frequency cutoff at around 50 to 100 GHz (see Fig. 3).
Now, what can be done with that? It is important to mention that the RF signal created with the laser source is inherently very stable. An RF signal can be created on one point, and a coherent copy of that signal can be created at a very different point that is connected with the original source by an optical fiber, which is much easier to handle and less lossy than an RF waveguide. The optical output can be sent over hundreds of meters, even in harsh environments, allowing the RF signal to be generated where it is needed (see Fig. 4). Both RF signals carry the high quality of the laser signal—that is, extremely low jitter and a high phase stability (see Fig. 5).
The RF signal could be selected in the X-band, which spans 8 to 12 GHz. This band is used not only for radar, but also in a variety of communication systems. Deep-space missions such as the old Voyager missions as well as current Mars missions such as Curiosity, use the X-band for communication. Some of its sub-bands are reserved for military satellites. Regular communications satellites make more use of the so-called Ku (“K under”) band at 12 to 18 GHz, while SpaceX’s Starlink satellites operate at around 24 GHz. Whatever RF frequency is needed can be produced from a laser source at an unprecedented quality.
Outlook
A new generation of ultrashort-pulsed lasers is coming to the market. With its monolithic resonator, this type of laser delivers very stable pulses from a rugged box. It is promising for a number of applications where its low jitter and high phase stability can be exploited. These may be in timing distribution, precision radar, or in communication technology, where photonics replaces electronics in more and more functions. This technology will also be used in space applications, for which many related tests on this laser source have been completed successfully.
REFERENCE
1. A. Khilo et al., Opt. Express, 20, 4, 4454–4469 (2012); https://doi.org/10.1364/oe.20.004454.
Search
Popular posts
- Menhir Photonics partner, CyberRidge shortlisted for Photonics Frontiers Award
- COHERENT OPTICAL COMB CHARACTERIZATION
- Unlock new possibilities: With new high-performance GHz laser
- Menhir Photonics: First Industrial GHz Laser at 1030 nm
- Photonic synchronization: navigating the future of telecommunications infrastructure
Popular tags